Overview
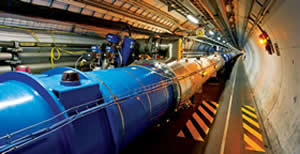
The Large Hadron Collider (LHC) is the largest, most complex machine ever built. Producing high energy collisions between subatomic particles to investigate matter, force and energy at the most fundamental level, it gives us a deeper understanding of the fabric of the universe.
This resource enables you to:
-
get inside the workings of the LHC and investigate how charged particles are accelerated to almost the speed of light
-
understand key terms and concepts about the physics of the fundamental particles that make up matter—particle physics
-
complete tasks that complement the NSW Physics Syllabus
-
analyse real data collected by the LHC ATLAS detector
-
visit virtually CERN scientists working at the ATLAS detector on the Large Hadron Collider, CERN—Particle physics with the masters at ATLAS CERN.
This resource can be used as a standalone extension exercise for students to work through, as a project based learning exercise with a major work due at the end, as a collaboration with the Hands on Particle Physics at ATLAS CERN program or a combination of these ideas.
If this resource will be used in conjunction with the International Masterclass ensure that the appropriate registration has taken place by reading the set up information in the ‘Information for teachers’ section.
Inside the Large Hadron Collider
Particle physicists want to better understand the universe and the fundamental particles and forces of the basic makeup of matter.
In the mid-twentieth century physicists developed hypotheses about the mechanism that gives mass to particles. Like, why do particles have the measured mass, and why do some not have mass? The best explanation was given in 1964 by Peter Higgs4, who assumed the existence of a particle, the boson which bears his name, which is the mediating particle of the Higgs field. This field gives mass to each particle it interacts with.
To test this and other hypotheses they built the Large Hadron Collider5 (LHC).
With this machine scientists can probe deeply into the origin of mass, dark matter, and new phenomena such as supersymmetry or extra dimensions.
Watch the CERN Experiment to get an overview of the LHC, and then view the Large Hadron Rap (4 mins 48 secs) for a creative explanation of the collider.
The LHC was designed to accelerate hadrons, like protons, at almost the speed of light. Visit 'How an accelerator works'6.
As these hadrons collide, they smash to bits, spit out their constituent particles and also create new particles. It is the energy of the colliding particles that is converted into new particles. Although most of the resulting particles do not last for long, they do leave tracks in the detectors incorporated into the accelerator. The tracks are stored as data in the detector’s computing grid. It is these tracks that can be interpreted. See 'How a detector works'7.
And this is what gets particle physicists excited using the Compact Muon Solenoid (CMS) and ATLAS (A Toroidal LHC Apparatus) detectors, ‘two of a kind, they are looking for whatever new particle they can find’. We will focus on ATLAS which was designed to cover the widest possible range of investigations. Its main objectives are to discover the Higgs boson (which it did on 4 July 2012), to confirm (or not) the principle of supersymmetry, to study matter and antimatter, the formation of dark matter and to search for extra dimensions and new phenomena.
Take the virtual tour of the ATLAS detector on CERN’s ATLAS8 page. A diagram of its structure allows you to see a cut section.
Note the position of detecting structures comprising ATLAS
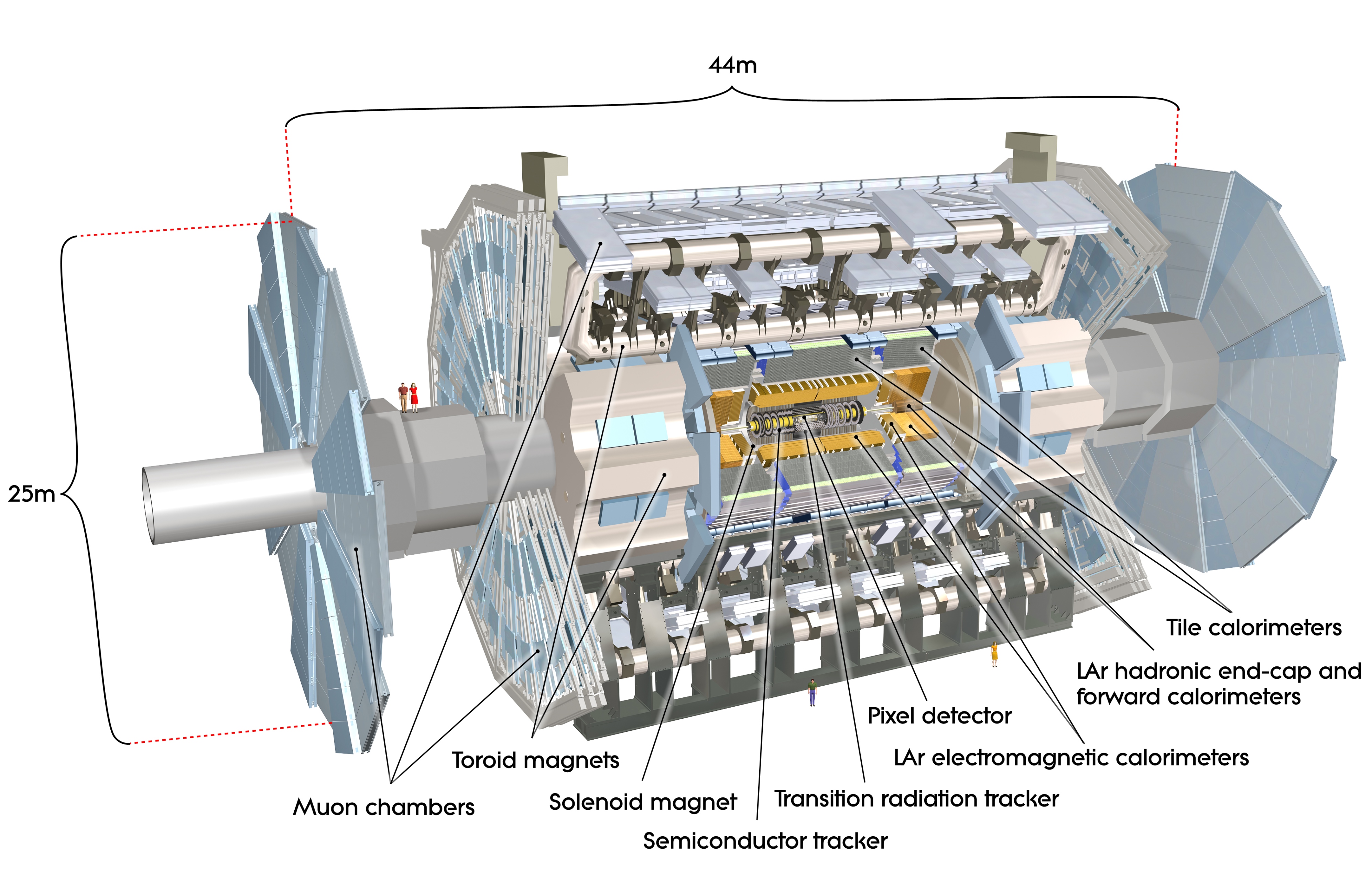
©2 CERN3
The ATLAS detector consists of a series of concentric cylinders mounted so that the beams collide in the centre. The particles resulting from the collision pass through the detectors in turn and either stop at one of them or continue on their way out of the detector.
The different layers allow us to identify the particles and measure their direction, charge, momentum, and energy. An example of how different particles interact with different layers of the detector is shown in the illustration ‘Interaction of particles with the ATLAS detector’.
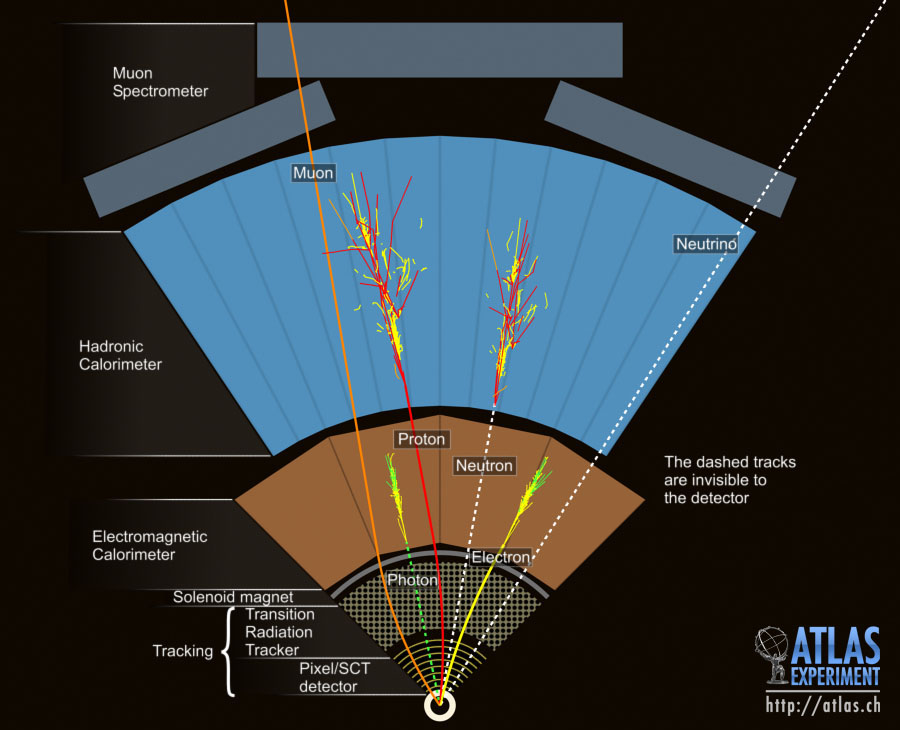
©2 CERN3
In the ‘Analyse ATLAS data’ section below, you will see similar representations of the ATLAS detector when you prepare to analyse decay particles in proton-proton collision ‘events’.
ATLAS is comprised of individual detectors:
The detector is located in the centre of the device and consists of three different sub-detectors, all designed to detect charged particles. The neutral particles (e.g. photons) pass through the detectors unnoticed. All charged particles interact with the detector, but exit with about the same energy and direction as before entering.
In the innermost part are high-resolution semiconductor detectors while the outer parts are gas detectors. All elements are mounted inside the central solenoid which creates a magnetic field with 2 Tesla intensity. The internal diameter of the detector is 1.15 m while its total length is 7m.
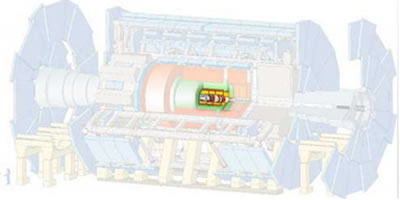
©2 CERN3
Calorimeters measure the energy of both charged and neutral particles. They consist of absorbers and active parts. The collision of particles with the absorbers creates a shower of secondary particles that are collected and recorded by the sensors. The energy of the initial particle in most cases is absorbed by the calorimeter. Because of this, the calorimeters are located outside the inner detector, so that the track of the charged particle is recorded before being absorbed by the calorimeter.
The ATLAS calorimeters consist of two different sections:
-
The electromagnetic calorimeter (green area) in which the hadrons leave a small portion of their energy. In contrast, the electromagnetic calorimeter absorbs the full energy of e+, e- and photons.
-
The hadronic calorimeter (orange area) measures the total energy of hadrons (like protons, neutrons).
Only muons (and neutrinos) have the ability to penetrate the detector first, and then the calorimeters, leaving little energy, and continue to the muon chambers.
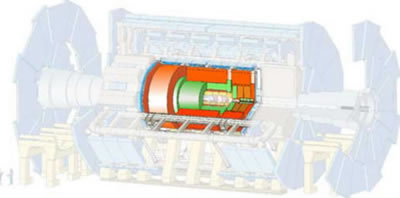
©2 CERN3
The muon chambers consist of thousands of tubes which detect charged particles positioned in the magnetic field created by huge superconducting toroidal coils.
The muons are particles like electrons, but they are about 200 times heavier. They are the only charged particles that can pass through the calorimeters without losing very much and reach the muon chambers located in the outside layer of ATLAS. The muon chambers measure the momentum and the trajectory of muons that cross them with great precision.
The only particles that pass through ATLAS undetected are neutrinos.
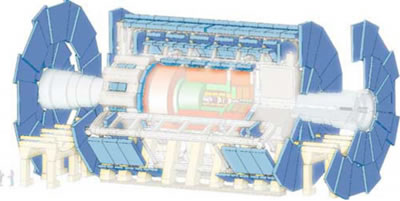
©2 CERN3
Watch the video, ‘The LHC Magnets’ (56 secs) for more details.
ATLAS uses two types of magnets, solenoid and toroidal with eight coils each. The detector is in a strong magnetic field which bends the trajectories of charged particles. The magnetic field generated by four different magnets: three toroidal (one large central and two smaller pieces in front) and a solenoid. Positively and negatively charged particles are curved in opposite directions from the same magnetic field. The radius of curvature and direction of the particles is used to determine the momentum and the charge of particles.
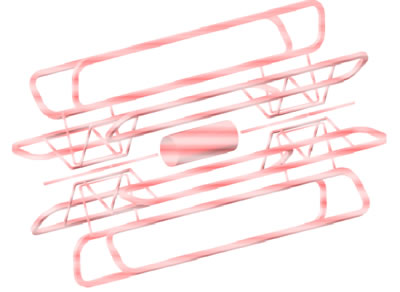
©2 CERN3
You can see the large toroidal magnets being incorporated in this two minute video of the 'Construction of the ATLAS detector'.
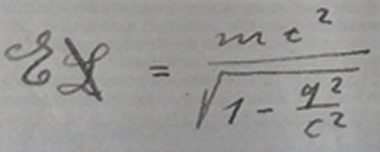
Wikimedia Commons9Public domain10
Energy is a critical quantity in understanding particle physics.
Particle physics measures energy in electron volts rather than Joules due to the tiny particles involved.
An electron gains an electron volt when it accelerates across a potential difference of 1 volt.
As voltage is defined as V= E/q, the energy gained by a charge when accelerated across a voltage V is given by E = qV and hence 1eV = 1.6 x 10-19 x 1 = 1.6 x 10-19 J
1eV = 1.6 x 10-19 Joules
Watch 'The electronvolt – A level physics' for an explanation of the electron volt and voltage.
Understanding the LHC experiments requires coming to grips with very small and very large quantities.
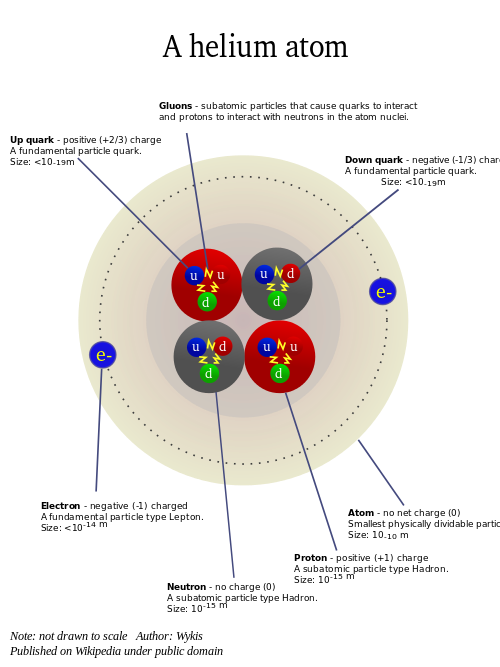
Wykis11 Public Domain10
A detailed accessible description of the internal structure of a helium atom.
The 'Scale of the Universe 2'12 (requires Flash) will provide you with useful size comparisons for many things in the universe.
The International System of units (SI) uses prefixes to specify the size of the quantity. See the SI units and prefixes table below.
International System (SI) units
Prefix
Symbol
Decimal
Scientific notation
yotta
Y
1,000,000,000,000,000,000,000,000
1024
zetta
Z
1,000,000,000,000,000,000,000
1021
exa-
E
1,000,000,000,000,000,000
1018
peta-
P
1,000,000,000,000,000
1015
tera-
T
1,000,000,000,000
1012
giga-
G
1,000,000,000
109
mega-
M
1,000,000
106
kilo-
k
1,000
103
hecto-
h
100
102
Deca-
da
10
101
—
—
1
100
deci-
d
0.1
10-1
centi-
c
0.01
10-2
milli-
m
0.001
10-3
micro-
μ
0.000 001
10-6
nano-
n
0.000 000 001
10-9
pico-
p
0.000 000 000 001
10-12
femto-
f
0.000 000 000 000 001
10-15
atto-
a
0.000 000 000 000 000 001
10-18
zepto-
z
0.000 000 000 000 000 000 001
10-21
yocto-
y
0.000 000 000 000 000 000 000 001
10-24
Protons and neutrons are believed to have diameters of approximately 10-15 m or 1 f (1 femto-metre), while the largest energy collision achieved to date in the LHC had an energy of 13 x 1012 eV of 13 TeV (13 Tera electron volts).
Mass in physics is usually measured in kilograms, but because particle physicists deal with such small masses they use atomic mass units (u) which is defined as 1/12th of the mass of a Carbon 12 atom.
1 u = 1.6605 x 10-27
Einstein showed that mass was a form of energy. Using his equation E = mc2 we can show that a gram of matter is equivalent to 9 thousand billion joules (90TJ) of energy and that an atomic mass unit is equivalent to 931.5MeV of energy.
1u = 931.5eV/c2
Therefore an electron can be said to have a mass of 9.10938356 x 10-31 kg or 0.000548579909u or an energy equivalence of 0.5109989461 MeV.
The LHC uses electric fields to accelerate particles to velocities close to the speed of light.
View this video on 'Electric fields'.
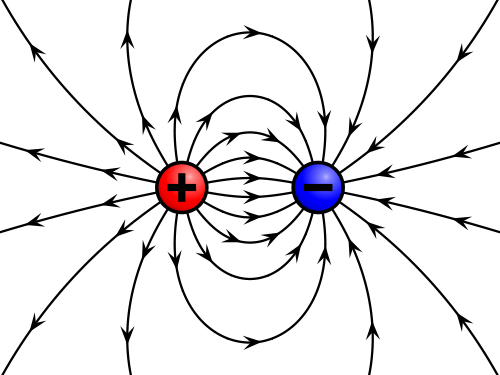
Geek313 CC BY SA 3.014
An electric field can be shown by drawing lines of force that show the direction that a positive charge would experience a force if placed at each point.
The direction of the electric field at a point in space is determined by placing an imaginary positive ‘test charge’ at the point. The direction of the force on the positive test charge is the direction of the electric field at that point.
We can show the electric field by drawing lines of force showing the direction a positive charge would experience a force if placed at each point.
Watch 'Electric field definition' for more information.
Electric fields surround charged objects
The strength of the field at a point is defined as the force that a unit positive charge would experience if it was placed at the point:
Formula: E = F/q |
Key: E: electric field strength F: force q: charge |
We can use this relationship to show that the units of the electric field are newtons per coulomb (NC-1). And we can rearrange this relationship to show that the force on a charge (q) place in an electric field of strength (E) is given by:
F = qE
We can make a simple charge accelerator by placing a voltage across parallel plates using two methods. See the diagram, right.
If the space between the plates is evacuated, a charge placed near the plate with the same polarity as the charge will be accelerated by the electric field towards the opposite plate.
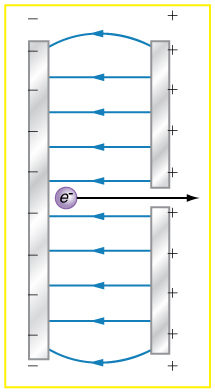
Accelerating charges between parallel plates
View the video 'Accelerating charges'.
Because the mass of protons and ions are so small, electric fields can accelerate charges rapidly. By directing charges around a circular path, and repeatedly passing them through an accelerating electric field, they can be accelerated to velocities near the speed of light.
For non-relativistic velocities we can calculate the final velocity of the charge using either Newton’s second law or conservation of energy.
When a charge is accelerated across a voltage (V) the energy gained by the charge is given by:
Energy = qV
This equation applies to relativistic and non-relativistic speeds so when particle physicists talk about energies of 40TeV they are referring to the energy that an electron would gain if it was accelerated across a potential difference of 40x1012 Volts.
Magnetic fields surround magnetic materials and electric currents where a force is experienced.
A magnet experiences a force when it is placed in a magnetic field.
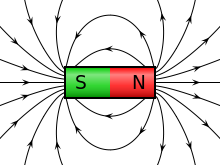
Geek317 CC BY-SA 3.018
Magnetic fields are called ‘B’ fields and the strength of the field is measured in Tesla. The earth’s magnetic field has a strength of approximately 50μT; the LHC uses magnetic fields as high as 8T.
Magnetic fields are directed from the north pole to the south pole of a permanent magnet. See the diagram, right.
Charges only experience a force in a magnetic field if they are moving and some component of their velocity is perpendicular to the magnetic field. If the charges are stationary or moving parallel to the magnetic field, they do not experience a force.
When a charge moves perpendicular to a magnetic field the magnetic field exerts a force given by:
F = qvB
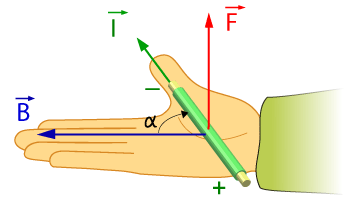
Jfmelero19 CC BY-SA 3.020
The direction of this force is perpendicular to the velocity of the charge in a direction given by the right hand palm rule.
A negative charge moving in a magnetic field would experience a force in the opposite direction to the force that a positive charge would experience. So, you can use your left hand to find the force direction when the moving charge is negative.
Magnetic fields are used to guide the high speed charged particles around the LHC.
Making a charge move in a circle
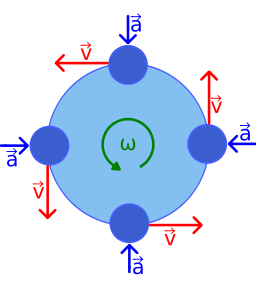
A detailed accessible description of uniform circular motion.
Because the magnetic force exerts a force that is perpendicular to the velocity of the charge the force acts as a centripetal force that will cause the charge to move in a circular path.
View the video 'Circular motion in a magnetic field'.
The radius of curvature of a charged particle moving perpendicular to a magnetic field is given by:
r = mv/qB
As the magnetic force exerts a centripetal force, we can use the centripetal force equation (Fc = mv2/r) to find the radius of curvature:
Fc = Fmagnetic
mv2/r = qvB
Hence, r = mv/qB
Einstein showed that if you were moving really fast and someone else was moving slowly, you would not agree on measurements of length, mass and time, as they are not absolute quantities. They will be different in different frames of reference.
At low velocities this impact of relativity is negligible and can be ignored. But when relative velocities approach the speed of light the effect of relativity on mass, length and time must be taken into account.
Watch the video below, ‘Special Relativity: Crash Course Physics #42’.
Einstein proved that if all rest frames are equivalent and the speed of light is an absolute constant, then an observer would see:
-
time run slow
-
length shrink in the direction of motion
-
mass increase in a frame moving with respect to the observer.
The amount that these quantities change can be calculated using Einstein’s equations of special relativity:
Time dilation formula: Length contraction formula: Mass dilation formula: |
Key: t = time l = length m = mass v = relative speed c = the speed of light = 3 x 108 ms-1 subscript 0 (0)= the quantities in the rest (proper) frame subscript v (v) = the moving frame |
Why the mass of a particle increases as its speed increases.
Watch 'Special relativity' for a more detailed explanation.
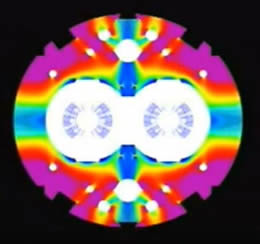
©2 CERN3
Superconductors play a special role at the LHC. The collider’s superconducting magnets use superconducting wire in their windings to keep the circular path of the particles constant when the particle’s mass increases as it approaches the speed of light.
The magnetic field has to be increased from 0.54 to 7.7 Tesla as the particles are accelerated from 450GeV to 6.5TeV.
The resistance in superconductors drops to zero because below the critical temperature the electrons in the superconductor interact as Cooper pairs23 existing in the same quantum state.
View these videos for more information:
Task: Discuss use of superconductors
Use the material in this section to learn about superconductors. Also research other uses of superconductors. Discuss the issues with superconductors, including its advantages and disadvantages of their use.
See Technology rubrics for any classroom24 for guidelines for interactive tutorials and adapt the guidelines for screencasts and vodcasts for your podcast. (A podcast will require less time than a screencast or vodcast.) Aim for an Outstanding ‘Grade’.
Standard Model of particle physics
In the last 50 years scientists have made a giant leap with the age old questions:
-
What are the ultimate building blocks of reality?
-
What are the forces that govern it?
Our modern understanding of what underpins the universe has been able to explain phenomena from the behaviour of atoms to how stars form. The name for this understanding is called the Standard Model of particle physics.
Task: Discuss key features of the Standard Model
Use the material in this section to learn about the Standard Model. After completing this section present your knowledge about the key features and components of the Standard Model of matter, including bosons, quarks and leptons. Identify issues with the model and include its advantages and disadvantages. You may collaborate on these digital works.
Two suggested versions:
-
Podcast or interactive tutorial that can accompany your other study material on the topic.
-
One minute podcast, or tutorial, that you can offer for posting on your school website so that a wider audience gains the benefit of your knowledge. Make sure that this version uses ordinary language, gives a broad view and touches on some concepts that the school community may have heard of but do not understand.
See Technology rubrics for any classroom24 for guidelines for interactive tutorials and adapt the guidelines for screencasts and vodcasts for your podcast. (A podcast will require less time than a screencast or vodcast.) Aim for an Outstanding ‘Grade’.
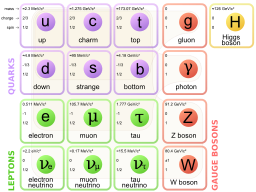
MissMJ25 CC BY 3.026
The Standard Model of particle physics describes the fundamental particles and forces of nature. Fascinatingly, ordinary matter is made up of only a few—electrons and ‘up’ and ‘down’ quarks.
The model includes many particles that only exist for tiny amounts of time.
The LHC was built to test the Standard Model and future models that will replace it. The predictions of the Standard Model, that have been tested, have all been verified.
Most physicists believe the model is still incomplete because it:
-
requires 20 experimentally determined factors to make it work
-
says nothing about dark matter and dark energy
-
fails to include the force of gravity.
Read more information about the particles of the Standard Model.
Learn more about the Standard Model by watching these videos:
-
The Standard Model of Particle Physics (Tobias Golling, CERN)
-
Theory of everything (The Standard Model)
-
Every force in nature (Theory of everything, part III) (Forces).
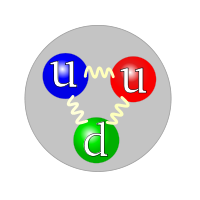
Arpad Horvath27 CC BY-SA 2.528
The Standard Model suggests that all the matter in the universe is made up from just three types of particles:
Quarks are charged particles that experience the strong nuclear force, the force that holds the nucleons together in the nucleus.
Particles made up of quarks are called hadrons:
-
baryons are hadrons with three quarks. Protons (see diagram on right, with two ‘up’ quarks and one ‘down’ quark) and neutrons are examples of baryons
-
mesons are hadrons consisting of quarks and antiquarks
Quarks interact via the strong force, which is so powerful that we never observe isolated quarks.
Leptons are particles that do not experience the strong force. Electrons and neutrinos are examples of leptons.
Watch 'Neutrinos – nature’s identity thieves?' to learn more about these particles.
Gauge bosons, such as photons, are called force mediating particles because they are the quanta of force fields (more on this later).
The Higgs boson was predicted by the Standard Model in the 1960s, but its existence was only confirmed by experiments at the LHC in 2012.
The Higgs particle is not a force mediating particle, but is still considered to be a boson as it is the quanta of a field that is responsible for giving particles their mass (inertia).
Watch a simple explanation of the Pauli Exclusion Principle in the 'Theory of Everything: What is Matter'.
Quarks and leptons are grouped together as fermions as they obey the Pauli Exclusion Principle (fermions cannot occupy the same quantum state).
Bosons do not obey the Pauli Exclusion Principle therefore can be placed in the same quantum state. This is why it’s possible to make lasers in which huge numbers of photons (bosonic particles) can be produced in the same quantum state.
Unstable particles
Most of the particles of the Standard Model are unstable and decay very quickly, before they can be detected.
Scientists can only directly detect electrons, muons, photons and hadrons.
Unstable particles are reconstructed from their decay products and the tracks they leave in the detectors.
Ordinary matter is made up of only a few electrons, and up and down quarks. The LHC may be able to tell us why.
Currently, physicists believe there are only four fundamental forces in nature. From weakest to strongest these are:
In the Standard Model these forces are mediated by force carrying particles called gauge bosons. The standard model encompasses all the forces except gravity. It is hypothesised that gravity has a force carrying particle. This particle has been given the name ‘graviton’. It must be different from the other force carrying particles. It is yet to be discovered.
Features of the particles that carry/mediate the fundamental forces
Force carrying particles |
Force |
Features |
photon |
electromagnetic |
|
W+ W- Z |
weak nuclear |
|
gluons (8 types) |
strong nuclear |
|
By colliding protons at higher energies and in higher numbers the LHC continues the exciting voyage towards new physics. This new physics will include the nature of dark matter. Watch this video to learn more about dark matter.
Contrasting normal matter and dark matter
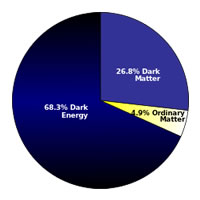
Szczureq32 CC BY-SA 3.022
Contrasting normal matter and dark matter
Blank cell |
Normal matter |
Dark matter |
Percentage of universe? |
5% |
85% Astrophysics observations detect its gravitational effect and indicate this percentage |
What it’s made of? |
Atoms made of:
|
We don’t know. We know very little about it That is why it’s called ‘dark’ matter |
Predictions |
Blank cell |
Made of Weakly Interacting Massive Particles—WIMPs for short |
If you want to know more about dark matter watch 'Complex dark matter'.
Gravity | extra space dimensions | string theory
Watch this video to learn more about gravity at the sub-atomic level. That is the quantum level.
Gravity at the microscopic quantum (minimum amount) scale
Ideas |
The Standard Model (SM) |
Beyond SM—extra space dimensions and string theories |
Gauge bosons are the carriers of fundamental forces |
Photon—electromagnetic force W+, W- and Z—weak force Gluon—strong force No explanation —gravitational force |
Hypothesised that a gauge boson, called a graviton (yet to be discovered) carries the gravitational force |
There is no description of gravity at the tiny quantum scale |
SM does not incorporate the general relativity theory of gravity. SM would expect gravity to be much stronger than it is. |
A graviton not yet observed |
Fundamental units |
Particles |
Strings (that is, all particles are ultimately the vibrations of tiny strings) |
Dimensions |
4 dimensional space-time |
10 or 11 space-time dimensions |
If LHC discovered… |
gravitons and microscopic black holes SM would need to be revised to include them |
gravitons and microscopic black holes they would support this theory |
What is expected of gravity? |
That it would be as strong as the other forces at this tiny scale… but it’s not |
A new law of gravity |
What it does or does not predict |
SM does not account for the accelerating expansion of the universe which is evidenced SM does not contain any ‘dark’ particle yet it is deduced from astrophysics observational |
Predicts extra space dimensions into which gravity can ‘escape’ and from which other Standard Model particles can’t escape |
String theories hypothesise vibrating springs—each vibration of the strings presents a different fundamental particle |
Particles are vibrations of open-ended strings that are attached to our three dimensional space like violin strings are attached to the instrument |
The graviton is the vibration of a closed loop, like a rubber band and because they are not attached they can leak into extra space dimensions, making gravity weak in our 3-dimensional space |
Watch this video on supersymmetry for more information.
Carriers of the weak force and the maker of mass
Many fundamental (indivisible) particles were discovered when larger particles collided inside particle accelerators, including the particles that carry/bring-about/mediate the weak force—W and Z bosons. And the one that gives all particles their mass — the Higgs boson.
Some particles are very short-lived and decay almost immediately to more stable particles.
Track massive particles when they are produced
In most proton collisions, the protons have gentle interactions, and the majority of the particles continue in the direction of the original particles (rather than perpendicular to the beam). But occasionally a few very massive particles are produced. If particles originate from the decay of a parent particle (the particle that gives rise to the new particle), their tracks should join together at the same original point, called a "vertex".
Most massive particles’ decays often give rise to several high-energy particles going out at large angles, more or less perpendicular to the original protons in the beam. For this reason LHC physicists measure the momentum and use it to pick up interesting events. By adding up the decay products which come from the same vertex they reconstruct the original "invisible" particle.
The mathematical representations of collisions and decay are highly complex. Richard Feynman33 developed a graphical representation to model these interactions.
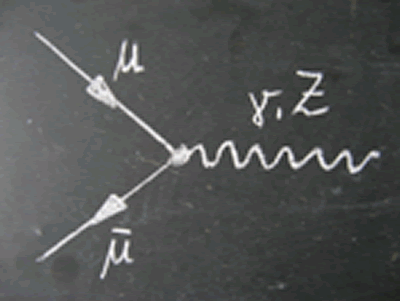
©2 CERN3
Feynman diagrams help you:
-
understand complicated interactions between particles through simple visual representations
-
understand interactions that occur in the detectors of the LHC
-
be prepared to analyse ATLAS data where we will study the Z and W bosons in particular.
Learn how to read Feynman diagrams
Feynman diagrams consist of space and time axes.
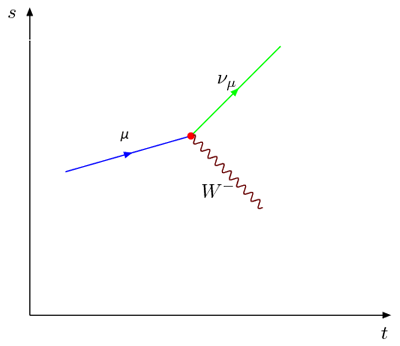
©2 CERN3
Interactions occur where the lines intersect (here: red dot). They also mark places where charge, momentum and energy must be conserved. This red dot represents the muon decay into a W- particle and a muon neutrino. The W- particle is called a messenger particle.
You can understand the time sequence from the horizontal t axis. The spatial sequence can be understood from the vertical axis. [Cosmic ray muons (μ) have a lifetime of 1/500 of a millisecond before they decay.]
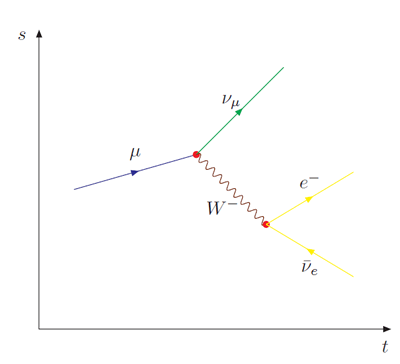
©2 CERN3
The second diagram shows a second interaction (second red dot), shows the creation of particles when the W- decays into an electron (e-) and an electron antineutrino ( ̅νe).
Leptons are straight lines with a little arrow in the middle of the line whereas the messenger particles of the weak (W, Z) and electromagnetic (γ) interactions are shown by wavy lines. Antileptons are always displayed with an arrow that points backward in time.
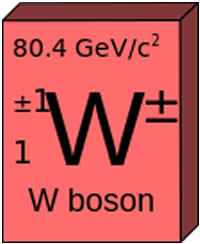
Lots of particles are created in a proton-proton collision (a so-called "event"). A key task of particle physicists is to search for specific elementary particles in these events then link them to the physical processes that might have produced them.
The W boson (carrier of the weak force) plays an important part in radioactive decay. The weak nuclear force is the fundamental force responsible for radioactive decay, for example the decay of Potassium-40.
Visit W-Path35 (Flash required) and select the green arrow on the image for an animation of how particle physicists ‘see’ beta decay happening in our bodies.
Practice reading beta decay with Feynman diagrams
During beta minus decay, an electron is emitted from an unstable nucleus when a neutron inside the nucleus transforms into a proton. An electron anti-neutrino is also produced. In the diagram below the:
-
Second down quark (d) transforms into a W- particle and an up quark (u).
-
W- particle decays into an electron and anti-neutrino.
©2 CERN3
During beta plus decay, a positron is emitted from an unstable nucleus when a proton inside the nucleus transforms into a neutron. An electron neutrino is also produced. In the diagram below the:
-
Second up quark (u) transforms into a W+ particle and a down quark (d).
-
W+ particle decays into a positron and a neutrino.
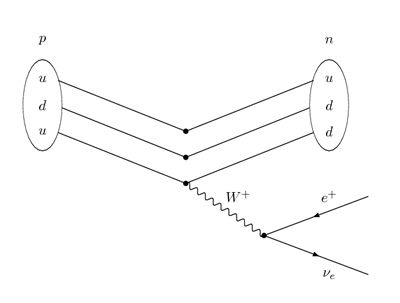
©2 CERN3
The W particle (also called W boson) is the particle most commonly produced in proton-proton collisions in the LHC. They almost immediately decay.
The W particle is heavy (80.4 GeV/c2) and decays almost immediately after it is produced:
-
2/3 of the time it decays to a quark-antiquark pair which appears as jets in the detector
-
1/3 of the time it decays to a lepton and a neutrino (the leptons, matter particles, can be an electron, a muon, or a tau
-
before the tau can be detected, it decays as well.
There are four processes producing W particles.
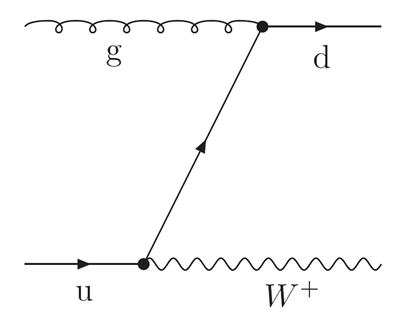
©2 CERN3
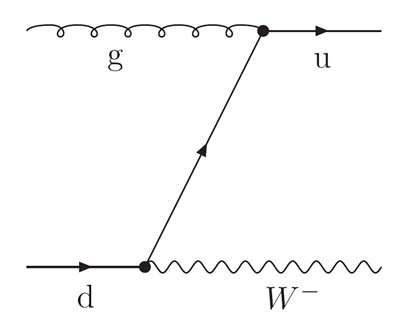
©2 CERN3
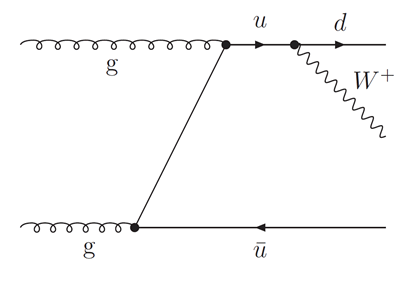
©2 CERN3
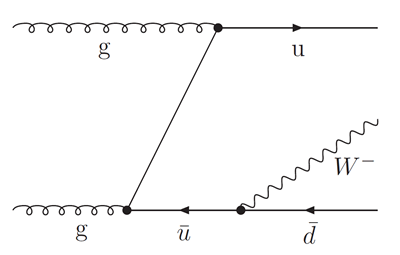
©2 CERN3
Signature events of W boson decay
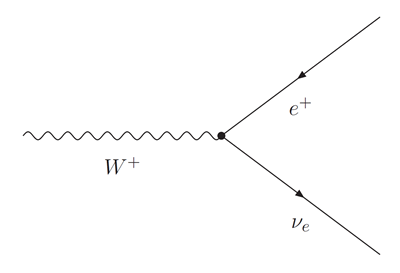
©2 CERN3
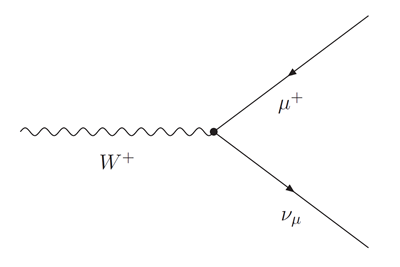
©2 CERN3
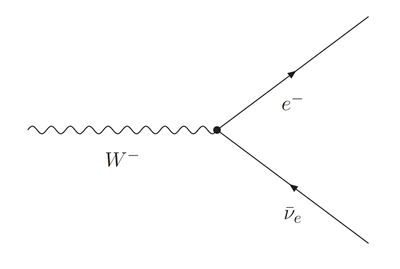
©2 CERN3
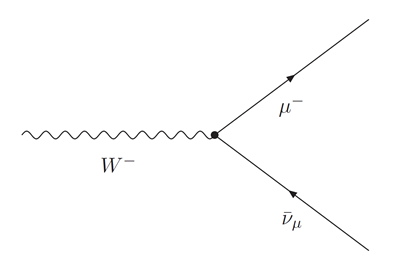
©2 CERN3
Other background events may occur, such as creation of Z0 particles.
Events, as in the Feynman diagrams above, leave signatures in the ATLAS detector. These are recorded as signal events in the ATLAS data samples.
Each signal event is an unambiguous indicator of a W particle which has existed for a very short time. All other events have to be categorised as background.
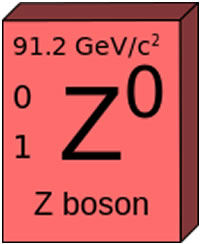
MissMJ36 CC BY 3.018
Z bosons:
-
are heavy, yet the tiniest of all particles
-
live for only 3 x 10-25 seconds before decaying, so they can only travel a short distance
-
travelling such short distances means that we cannot see the light of Z bosons. (If bosons did not have large mass, photons would probably not be massless. If photons were not massless they could not travel long distances. So common light (made of photons) would not exist
-
at high enough energies, the photon and the Z are closely connected.
-
-
carry the weak force (like W bosons)
-
the weak force has a very short range. It causes subatomic interactions like beta decay
-
-
allow neutrinos to interact among themselves
-
because neutrinos have no electrical charge they can’t interact amongst themselves via a photon
-
-
have no charge (charge = 0)
A: In the extreme conditions of supernova explosions. Z bosons are also thought to have been extremely common in the early universe.
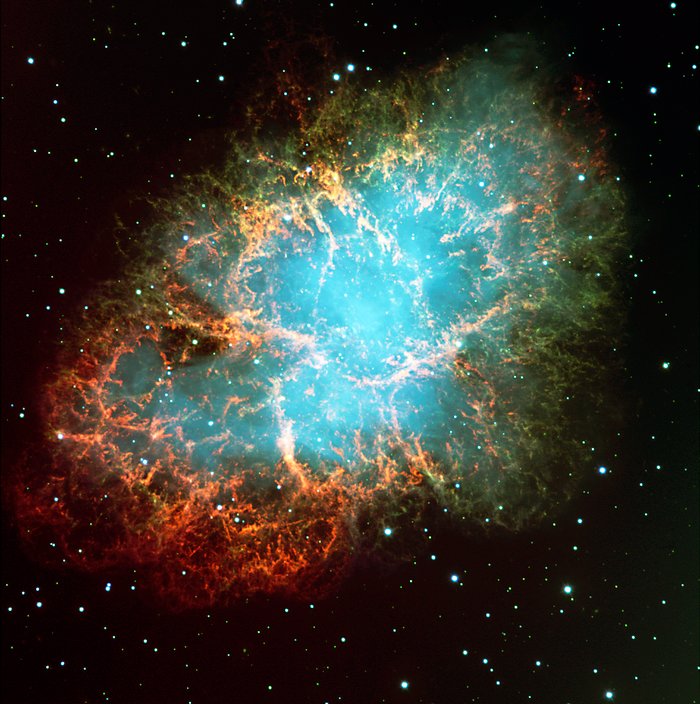
NASA 37 Public Domain10
In nature, charge is conserved. Since a Z boson is neutral the sum of the charges of its decay products must be zero charge. Therefore Z must decay into a particle and antiparticle pair.
The possible Z decays is divided between the groups of particles below according to conservation laws.
-
10% of the Z-decays, produce charged lepton-antilepton pairs. These include electron-antielectron (positron), muon-antimuon, and tau-antitau pairs all are equally probable.
-
20% of Z boson decays produce a neutrino-antineutrino pair. LHC detectors can’t detect neutrinos directly. So they are detected by the loss of some energy and/or momentum after the collision.
-
70% of Z decays produce a quark-antiquark pair. These appear as particle showers called “jets” in the detector.
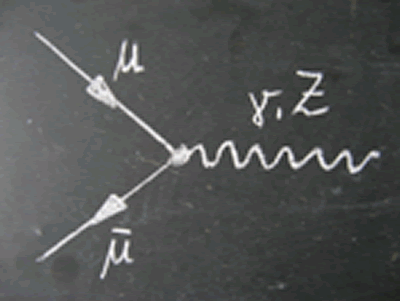
©2 CERN3
The Z' boson is a heavy partner of the Z boson (Z0).
Some theories beyond the Standard Model predict the need for a new weak force. These theories predict that the production and decay of the Z' boson will be very similar to that of the ordinary Z boson.
Using a pencil and paper, try your hand at drawing a Feynman diagram for
-
Z1 boson decay to an electron (e-) and a positron (e+)
-
Z1 boson decay to a muon (μ-) and an antimuon (μ+)
Visit Z' boson38 and select the hand-drawn Feynman diagrams on that page to check your Feynman diagrams.
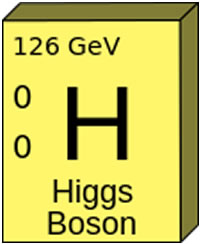
In July 2012, CERN announced the ATLAS experiment had discovery of a new boson. It had a mass of roughly 125 GeV. This is the mass of the hypothesised Higgs boson, which was predicted to make the Standard Model complete. It needed a something that gave particles their mass - the Higgs boson.
The Standard Model predicts how often the Higgs boson is produced in particle collisions and how it decays into known particles. It also predicts the Higgs boson’s spin which has been confirmed for the newly discovered particle. Using the ‘like a duck’ reasoning—if it looks like a duck, swims like a duck, and quacks like a duck, then it probably is a duck—the LHC had discovered the Higgs boson.
The Higgs boson is very heavy, even heavier than the Z boson.
Watch 'What is a Higgs Boson?' to get a better handle on the Higgs particle and its field.
Like the Z boson, it is unstable and has a very short lifetime. It decays almost immediately and therefore travels only a very tiny distance. Because of this, there is no chance of directly detecting it in ATLAS or any other particle detector. What can be detected are the Higgs boson decay particles.
View the Higgs particle page40 (select the Feynman diagrams at Signal vs Background) for representations of the production and decay of the Higgs particle and one background event, the production of a heavy top quark pair.
When protons travel at almost the speed of light, as they do in the LHC, they gain very large energies. Because of these large energies, when the protons collide all types of particles are emitted and also created:
Q: What is emitted or created?
A: Particles that ordinary matter is made of, and other particles that only existed just after the Big Bang.
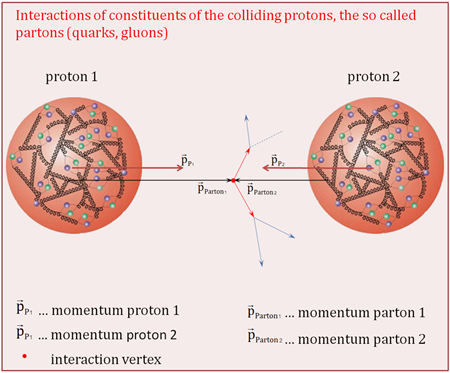
©2 CERN3
Inside each proton you can find a ‘sea’ of quarks and gluons. We say that a proton consists of three quarks, but it also has a whole bunch of ‘sea’ or ‘virtual’ quarks and anti-quarks stemming from gluons.
The new particles are usually much heavier than the original colliding particles. All the energy put into the collision can come out as mass instead of energy.
E=mc2
Energy and momentum are conserved.
The ATLAS detector is a sort of digital camera, recording what happens when protons collide (events). After collision, as the spat-out particles cross the ATLAS detector, they leave electronic signals or footprints.
To analyse data collected by the ATLAS detector we need to analyse these footprints to identify short-lived particles like the Z boson and Higgs boson.
Select the two video simulations (Flash required) on the 'Identifying Events page'41 to see how the ATLAS detector ‘sees’ proton collisions.
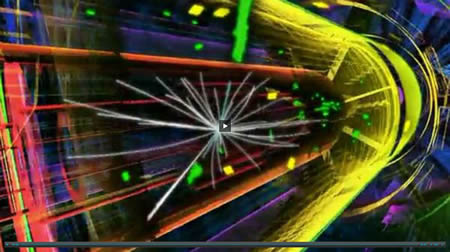
Researchers from the University of Witwatersrand, Johannesburg analysed CERN data and think they have seen 'Glimpses of the Madala boson: have we detected the dark Higgs?'42
Read the article, and then discuss why scientists can have different interpretations of results.
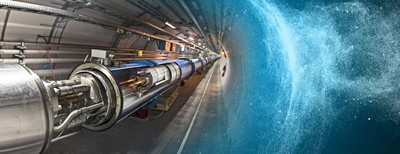
Analyse Atlas data
Screenshot © 2011 S.V University of Athens18
If you are committed to participating in the Particle physics with the masters at ATLAS CERN be sure to make your booking at least two weeks in advance. See Information for teachers section.
TASK:
Join the HY.P.A.T.I.A Q&A forum
Use HY.P.A.T.I.A43 online to identify tracks of particular particles that are produced when hadrons collide at high energies in the ATLAS detector:
-
Access help via the HY.P.A.T.I.A help guide for each exercise.
-
Use the Start page of HY.P.A.T.I.A online to guide you through the activities.
To join the 'Particle physics with the masters at ATLAS CERN' course you will need to work with your teacher to set up the Masterclass. This function is not yet availble.
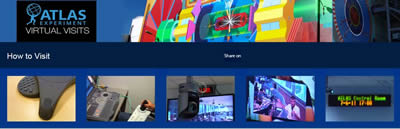
Information for teachers
Much of the depth of knowledge and understanding potentially acquired via the Masterclass is beyond the senior physics curriculum. It is included to allow for the deep understanding that provides a genuine masterclass where students, already at the top of the game, can interact significantly with the master.
Accessibility – IMPORTANT NOTE
This resource includes many equations and mathematical expressions that cannot be read by a screen reader. Some of these have been converted into images and have alt text that will be read as the correct wording by a screen reader but may present issues for someone with low vision who needs to increase the screen size hugely.
In the downloadable word documents the equations, where possible, have been created in such a way that they can be copied into STEMReader44 and converted into Mathml which will then be read by the build in screen reader in STEMReader.
Students with a visual disability will need assistance to fully engage with this resource. For schools within the Department of Education NSW you may need to access the Itinerant support teachers (hearing or vision)45 for your region through the ‘Ed services page46’.
Video conference – IMPORTANT NOTE
A virtual visit with ATLAS CERN is free. It is not free of commitment, if you wish to commit you will need to set up a Masterclass. This function is not yet availble.
Video conference opportunities with ATLAS CERN will be available soon through DART connections.
Aim of LHC physics masterclass
Its aim is to provide users with the opportunity to:
-
Build their physics knowledge and understanding so that the Particle physics with the masters at ATLAS CERN can be a genuine case of particle physics masters honing the users already deep understanding. Thus providing significant and genuine experience with working scientists at the forefront of their field.
-
Experience real scientific work by analysing real ATLAS data.
-
Communicate with working scientists to improve users’ scientific data analysis and understand the physics principles that allow scientists to draw conclusions and new insights from the data.
-
Meet curriculum outcomes in a meaningful way through participating in a cutting-edge real world context.
Ideas for using LHC physics masterclass with students
-
Use LHC physics masterclass for project based learning. The teacher may wish to design a culminating task for this purpose. It could require the completion of the tasks, data analysis and Particle physics with the masters at ATLAS CERN visit contained within the resource. Such a culminating task could be a collaborative task. Collaboratus: resource model47 can assist student groups to develop different collaborative products. Different groups of students could for example design and develop different types of products that demonstrate some aspect of their experience for a genuine purpose like science communication. Students could engage their creativity to develop ideas, decide on some, then team with other students interested the various ideas. The curriculum based tasks completed during the LHC physics masterclass could form the basis of a group’s vodcast for example or other communication format. What is Cooperative Learning: More than Just Working in Groups48 may assist.
-
Undertake the relevant sections of LHC physics masterclass during the year when relevant content or option is being covered. The Virtual Visit can be scheduled for a time when all curriculum content areas have been completed.
-
Use a flipped classroom approach where students do the learning outside school in preparation for working during class on tasks that require this preparation and perhaps some coaching from the teacher. This idea can also be combined with idea 2.
-
To revise and apply concepts covered earlier in the senior curriculum and develop students abilities to apply their knowledge to unfamiliar situations.
The resource supports students in Stage 6 Physics. The resource is designed to meet the skills and knowledge and understanding of the 2002 and 2017 NSW syllabuses. Teachers should integrate the resource in their teaching and learning programs and differentiate where required to meet the needs of individual students.
The following material is from the Physics Stage 6 Syllabus (Amended October 2002) © NSW Education Standards Authority49 (NESA) for and on behalf of the Crown in right of the State of New South Wales, 2012.
Possible Prescribed Focus Areas
H1. evaluates how major advances in scientific understanding and technology have changed the direction or nature of scientific thinking
H2. analyses the ways in which models, theories and laws in physics have been tested and validated
H3. assesses the impact of particular advances in physics on the development of technologies
H4. assesses the impacts of applications of physics on society and the environment
H5. identifies possible future directions of physics research
H9. explains the effects of electric, magnetic and gravitational fields
-
9.2 Space
-
4. Current and emerging understanding about time and space has been dependent upon earlier models of the transmission of light
-
Students
-
solve problems and analyse information using:
-
-
E = mc2
Time dilation formula: Length contraction formula: Mass dilation formula: |
Key: t = time l = length m = mass v = relative speed c = the speed of light = 3 x 108 ms-1 subscript 0 (0)= the quantities in the rest (proper) frame subscript v (v) = the moving frame |
-
9.4 From Ideas to Implementation
-
1. Increased understandings of cathode rays led to the development of television
-
Students learn to
-
identify that moving charged particles in a magnetic field experience a force
-
identify that charged plates produce an electric field
-
describe quantitatively the force acting on a charge moving through a magnetic field
-
discuss qualitatively the electric field strength due to a point charge, positive and negative charges and oppositely charged parallel plates
-
-
Students
-
solve problem and analyse information using F=qE
-
-
4. Investigations into the electrical properties of particular metals at different temperatures led to the identification of superconductivity and the exploration of possible applications
-
Students learn to
-
describe the occurrence in superconductors below their critical temperature of a population of electron pairs unaffected by electrical resistance
-
discuss the advantages of using superconductors and identify limitations to their use
-
-
H10. describes the nature of electromagnetic radiation and matter in terms of the particles
-
9.8 Option - Quanta to Quarks
-
4. An understanding of the nucleus has led to large science projects and many applications
-
Students learn to
-
identify ways by which physicists continue to develop their understanding of matter, using accelerators as a probe to investigate the structure of matter
-
discuss the key features and components of the standard model of matter, including quarks and leptons
-
-
H12. evaluates ways in which accuracy and reliability could be improved in investigations
-
12.3 gather information from secondary sources by:
-
e) identifying practising male and female Australian scientists, and the areas in which they are currently working and information about their research
-
-
12.4 process information to:
-
b) identify and apply appropriate mathematical formulae and concepts
-
H14. assesses the validity of conclusions drawn from gathered data and information
-
14.1 analyse information to:
-
f) use models, including mathematical ones, to explain phenomena and/or make predictions
-
-
14.2 solve problems by:
-
c) using identified strategies to develop a range of possible solutions to a particular problem
-
H16. justifies positive values about and attitude towards both the living and non living components of the environment, ethical behaviour and a desire for critical evaluation of the consequences of the applications of science
The following material is from the Physics Stage 6 Syllabus (2017) © NSW Education Standards Authority50 (NESA) for and on behalf of the Crown in right of the State of New South Wales, 2012.
PH12-13 explains and analyses the electric and magnetic interactions due to charged particles and currents and evaluates their effect both qualitatively and quantitatively
-
Subtopic: Charged Particles, Conductors and Electric and Magnetic Fields
-
Inquiry question: What happens to stationary and moving charged particles when they interact with an electric or magnetic field?
-
Students:
-
investigate and quantitatively derive and analyse the interaction between charged particles and uniform electric fields, including: (ACSPH083)
-
acceleration of charged particles by the electric field (F ⃗=ma ⃗ ,F ⃗=qE ⃗ )
-
model qualitatively and quantitatively the trajectories of charged particles in electric fields and compare them with the trajectories of projectiles in a gravitational field
-
analyse the interaction between charged particles and uniform magnetic fields, including: (ACSPH083)
-
acceleration, perpendicular to the field, of charged particles
-
the force on the charge (F ⃗=qv ⃗B ⃗sinθ)
-
compare the interaction of charged particles moving in magnetic fields to:
-
the interaction of charged particles with electric fields
-
other examples of uniform circular motion (ACSPH108)
-
PH12-14 describes and analyses evidence for the properties of light and evaluates the implications of this evidence for modern theories of physics in the contemporary world
-
Subtopic: Light and special relativity
-
Inquiry question: How does the behaviour of light affect concepts of time, space and matter?
-
Students:
-
investigate the evidence, from Einstein’s thought experiments and subsequent experimental validation, for time dilation (t=t_0/√(1-v^2/c^2 )) and length contraction (l=l_0 √(1-v^2/c^2 )) and analyse quantitatively situations in which these are observed, for example:
-
evidence from particle accelerators
-
describe the consequences and applications of relativistic momentum with reference to:
-
p_v=mv/√(1-v^2/c^2 )
-
-
the limitation on the maximum velocity of a particle imposed by special relativity (ACSPH133)
-
Use Einstein’s mass–energy equivalence relationship (E=mc2) to calculate the energy released by processes in which mass is converted to energy, for example: (ACSPH134)
-
particle–antiparticle interactions, eg positron–electron annihilation
-
PH12-15 explains and analyses the evidence supporting the relationship between astronomical events and the nucleosynthesis of atoms and relates these to the development of the current model of the atom
-
Subtopic: Deep inside the Atom
-
Inquiry question: How is it known that human understanding of matter is still incomplete?
-
Students:
-
analyse the evidence that suggests:
-
that protons and neutrons are not fundamental particles
-
the existence of subatomic particles other than protons, neutrons and electrons
-
investigate the Standard Model of matter, including:
-
quarks, and the quark composition hadrons
-
leptons
-
fundamental forces (ACSPH141, ACSPH142)
-
investigate the operation and role of particle accelerators in obtaining evidence that tests and/or validates aspects of theories, including the Standard Model of matter (ACSPH120, ACSPH121, ACSPH122, ACSPH146)
-
PH11/12-4 selects and processes appropriate qualitative and quantitative data and information using a range of appropriate media
-
Students:
-
select qualitative and quantitative data and information and represent them using a range of formats, digital technologies and appropriate media (ACSPH004, ACSPH007, ACSPH064, ACSPH101)
-
apply quantitative processes where appropriate
-
PH11/12-5 analyses and evaluates primary and secondary data and information
-
Students:
-
derive trends, patterns and relationships in data and information
-
assess the relevance, accuracy, validity and reliability of primary and secondary data and suggest improvements to investigations (ACSPH005)
-
PH11/12-6 solves scientific problems using primary and secondary data, critical thinking skills and scientific processes
-
Students:
-
use modelling (including mathematical examples) to explain phenomena, make predictions and solve problems using evidence from primary and secondary sources (ACSPH006, ACSPH010)
-
use scientific evidence and critical thinking skills to solve problems
-
This project targets 21st century learning skills51 including the following.
Skill |
Guiding questions |
Knowledge construction |
|
Skilful communication |
|
Real world experience |
|
Critical thinking |
|
The article ‘What is co-operative learning: More than just working in groups’48 identifies five key elements that differentiate cooperative learning from simply putting students into groups: positive interdependence, individual accountability, face-to-face interaction, interpersonal and small group social skills, and group processing.
For more information see the Collaboratus: resource model47 and look for the Collaboratus template in Google Sites (DoE only).
Ensure that students practice good citizenship by providing correct attribution and copyright information for images.
Useful links:
This resource was developed through collaboration to support the department’s Rural and Remote Blueprint for Action.
Contributors:
-
Dr Mark Butler - Content writing (much particle physics content was adapted from existing CERN material)
-
ARC Centre of Excellence for Particle Physics at the Terascale55 (CoEPP) physicists - Dr Caroline Hamilton, Communications and Outreach
-
ATLAS experiment56 at CERN57 physicists - Dr Steve Goldfarb and Professor Christine Kourkoumelis
-
The International Particle Physics Outreach Group58 (IPPOG) scientists supporting students’ online communication to Analysis ATLAS data - Professor Christine Kourkoumelis, Dr Stelios Vourakis, Dr Steve Goldfarb, Dr Caroline Hamilton, Associate Professor Archil Kobakhidze, Dr Shantha Liyanage
-
Brett McKay (Kirrawee High) - editing, testing and advice.
Links
- https://home.cern/topics/large-hadron-collider
- http://copyright.web.cern.ch/
- http://home.cern/
- https://en.wikipedia.org/wiki/Peter_Higgs
- http://home.cern/topics/large-hadron-collider
- http://home.cern/about/how-accelerator-works
- http://home.cern/about/how-detector-works
- http://home.cern/about/experiments/atlas
- https://commons.wikimedia.org/wiki/File:E_mc_2_IMG_0859.jpg
- https://creativecommons.org/publicdomain/mark/1.0/
- https://commons.wikimedia.org/wiki/File:Explained_Atom.svg
- http://htwins.net/scale2/
- https://commons.wikimedia.org/wiki/File:VFPt_dipole_electric_manylines.svg
- https://creativecommons.org/licenses/by-sa/3.0/
- https://cnx.org/license
- https://creativecommons.org/licenses/by-sa/4.0/
- https://commons.wikimedia.org/wiki/File:VFPt_cylindrical_magnet_thumb.svg
- https://creativecommons.org/licenses/by/3.0/deed.en
- https://commons.wikimedia.org/wiki/File:Regla_mano_derecha_Laplace.svg
- https://creativecommons.org/licenses/by/3.0/
- https://commons.wikimedia.org/wiki/File:Uniform_circular_motion.svg
- https://creativecommons.org/licenses/by-sa/3.0/deed.en
- http://viewpure.com/ryTN0PFip3w?start=0&end=0
- https://spark.adobe.com/page/QAdgM/
- https://commons.wikimedia.org/wiki/File:Standard_Model_of_Elementary_Particles-id.svg
- https://creativecommons.org/licenses/by/3.0/au/
- https://commons.wikimedia.org/wiki/File:Quark_structure_proton.svg
- https://creativecommons.org/licenses/by-sa/2.5/deed.en
- https://en.wikipedia.org/wiki/Gravity
- https://en.wikipedia.org/wiki/Electromagnetism
- https://en.wikipedia.org/wiki/Weak_interaction
- https://commons.wikimedia.org/wiki/File:DMPie_2013.svg
- https://en.wikipedia.org/wiki/Richard_Feynman
- https://commons.wikimedia.org/wiki/File:W_boson.svg
- http://atlas.physicsmasterclasses.org/en/wpath.htm
- https://commons.wikimedia.org/wiki/File:Z_boson.svg
- https://www.nasa.gov/multimedia/imagegallery/image_feature_1604.html
- http://atlas.physicsmasterclasses.org/en/zpath_zprime.htm
- https://commons.wikimedia.org/wiki/File:Higgs_boson.svg
- http://atlas.physicsmasterclasses.org/en/wpath_higgs.htm
- http://atlas.physicsmasterclasses.org/en/zpath_ereignis.htm
- https://cosmosmagazine.com/physics/reported-glimpses-of-the-madala-boson-have-we-detected-dark-higgs
- http://hypatia.iasa.gr/en/
- https://stemreader.org.uk/download-stemreader/
- https://education.nsw.gov.au/disability-learning-and-support/programs-and-services/itinerant-support-teachers#Itinerant1
- https://app.education.nsw.gov.au/school-services-contacts/
- https://schoolsequella.det.nsw.edu.au/file/23d078e5-d165-4942-8d28-4bc45759869b/1/Collaboratus-guide.zip/index.htm
- http://serc.carleton.edu/sp/library/cooperative/whatis.html
- http://www.boardofstudies.nsw.edu.au/syllabus_hsc/physics.html
- http://syllabus.nesa.nsw.edu.au/physics-stage6/
- https://education.microsoft.com/GetTrained/ITL-Research
- http://www.digitalcitizenship.nsw.edu.au/
- http://creativecommons.org.au/
- http://www.smartcopying.edu.au/information-sheets/schools/students-and-copyright
- http://www.coepp.org.au/
- http://atlas.cern/
- https://home.cern/
- http://ippog.web.cern.ch/